“I’m a survivor, I’m not gonna give up… or How do African midge larvae survive without water?”
A fun and approachable review of research: Tagmazian, A 2020 ‘Alternative Splicing Regulation in Polypedilum Vanderplanki under Dehydration-rehydration Cycle’, Master thesis, HSE University, Moscow.
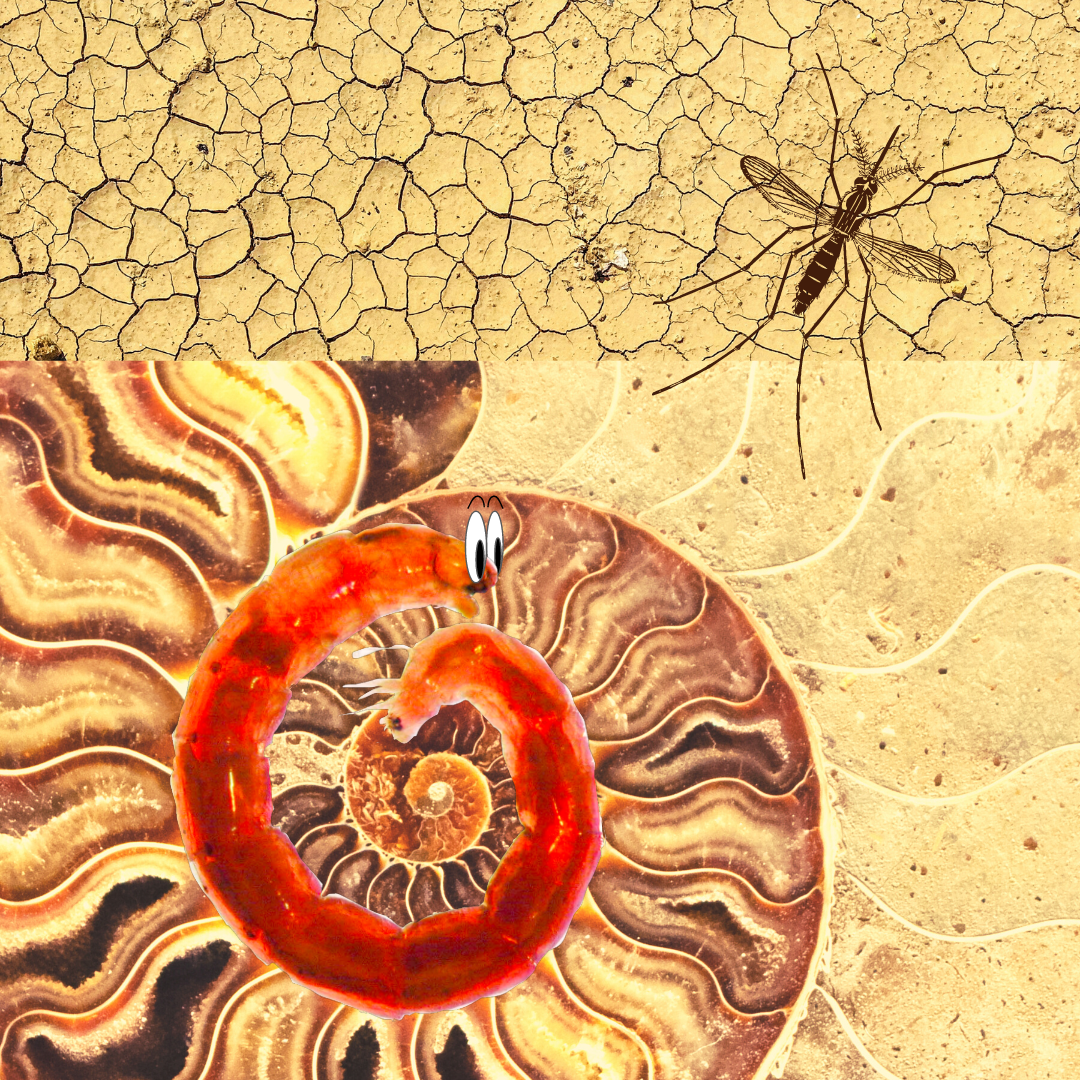
The Questions will be answered:
- How do Polypedilum vanderplanki larvae survive without water, and what role alternative splicing plays in this?
- Who is Polypedilum vanderplanki and why should I be interested in its survival?
- ‘Alternative splicing’ sounds really complicated. Is it possible to understand the main concept without a deep knowledge of molecular biology? (Spoiler alert: Yes, it’s possible!)
- Did the author find anything new?
A prequel of the research
It always fascinates me how scientists have made their discoveries because not everyone can come up with an idea like scientists did.
The discovery of the super-powered midge.
In 1949-1950, Dr. Frederic L. Vanderplank noticed a species of non-biting midge during his expedition in Africa whose larvae survived in dried mud in extreme temperatures and collected the first samples of it. Later British entomologist H. E. Hinton described the main characteristics of this species in his article and named it Polypedilum vanderplanki (Let’s call it PV).
What is its superpower?
PV lives a very common life compared to his closest relatives, however, its larvae have a ‘superpower’ or adaptation skill. In extremely high-temperature conditions, it can lose up to 97% of its body moisture, transforming into something that looks like a caramelized stick, and come back to an absolutely normal life when back in a favorable environment. Scientists have been exploring the boundaries of PV’s abilities for more than 50 years and have found out that larvae in a dried state can live for more than 17 years, be exposed to temporary high (103ºC = 217.4ºF) or low (-190ºC = -310°F) temperatures, radiation (7000 Gy, for comparison, 10 Gy are lethal for human) or immersion in 100% ethanol.
How can we use this superpower in the future?
PV is harmless to humans, as it doesn’t bite and (luckily) doesn’t spread illnesses. So why should we continue to study desiccation processes in this species? Because it has a fascinating adaptation skill and knowing the underlying processes could be used in agriculture and medicine, for example, in biomaterials storage. Just imagine, one day it could replace liquid nitrogen! Instead of unfreezing your samples, you can take a handful of dehydrated cells or tissues and just add water, as simple as instant noodles cooking.
My hypothesis.
Logically, for surviving without water the PV larvae body goes through significant transformations, which definitely include the rearrangement of proteins. Alternative splicing is one of the mechanisms underlying modifications of proteins’ sequences and functions (Alternative splicing in nutshells). In my research, I hypothesized that during the drying-recovery cycle PV larvae undergo a significant shift in alternative splicing and questioned what can regulate this shift.
Nice plots and what do they really mean
Everything starts from data
I had data from 5 different stages of the desiccation-rehydration cycle: normal state (d0), 24 hours of dehydration (d24), 48 hours of dehydration (d48), 3 hours of rehydration (r3), 24 hours of rehydration (r24). (Fig 1)
The splicing shift exists!
First of all, I checked if there was a significant change in splicing at all between the different conditions. In total, 1322 segments (including both exons and introns) significantly changed their inclusion in mRNA between the normal condition and at least one of the dehydration/rehydration stages. For example, segment N was included in mRNA on active larvae condition, but was cut off during all stages of dehydration, and included again in the rehydration process. So we can conclude that segment N was alternatively spliced during the dehydration. How else could the splicing of segments change between stages?
I separated the segment groups by 5 patterns based on the behavior of their inclusion/exclusion from the mRNA. (Fig 2) There are 3 major patterns in the plot: decreasing of segment inclusion during dehydration (clusters 1 and 3), increasing of segment inclusion during stages d24 and d48 (clusters 2 and 4), and decreasing of segment inclusion only on the stage r3 (cluster 5).
Now when we approve that a shift in alternative splicing between stages exists, we can move to the mystery question of alternative splicing regulation. Are there specific reasons why we observed those patterns, or are all the processes going nuts and starting to make mistakes because of heat shock?
I bet on cassette exons.
From the section ‘Alternative splicing in nutshells’ you could remember 4 different alternative splicing events: cassette exon, intron retention, alternative acceptor, and alternative donor splice site. First of all, I checked the distribution of those splicing events among the desiccation-recovery stages. Based on our data, during desiccation cassette exons were more often cut away from a sequence (not included in mRNA), while introns or their parts were frequently included. (Fig 3)
One really interesting explanation for the increase of intron retention under heat shock was presented in the paper by Shalgi, Reut, et al [1]. Authors discovered that RNAs with intron retention stay in the nucleus and were not used for protein synthesis during heat shock, they probably even were not spliced at all. Potentially the cell can use this pool of unspliced, almost ready-to-use, RNA during recovery after the stress. At the same time heat shock accelerates the splicing process, so ‘splicing control’ proteins just do not have time for dealing with cassette exons and therefore exclude them from a sequence [2].
Even though intron retention and alternative donor/acceptor events happen more often during the de/rehydration cycle, I continued my analysis only with cassette exons. Why? 1. In general, it was the simplest and better-studied way. (Yes, scientists also prefer to start from the easiest way.) 2. Inclusion/exclusion of a cassette exon brings the greatest functional changes for mRNA and synthesized proteins. 3. Methods of intron retention and alternative donor/acceptor events accurate detection is limited and just starting to develop.
Splice sites’ abnormalities? Not proven, not guilty.
To carry out the splicing, ‘splicing control’ proteins (Spliceosomes) bind to special regions in introns – splicing sites, and they prefer to have business only with strong splice sites. In general, alternative segments are surrounded by weaker splice sites compared to constant exons (which always stay included in mRNA). Is it possible that in the case of PV this rule was violated?
There are several well-known indicators of strong splicing sites. One by one I evaluated them and did not find any abnormalities. Thus, cassette exons in P. vanderplanki follow the common rule for the alternative splicing sites, and spliceosomes in PV larvae work the same way as for any other eukaryotic organism. What could then change spliceosomes’ minds and make them bind with weaker splice sites, and consequently cause alternative splicing events?
I can imagine only 2 reasons. First, due to heat shock, spliceosomes make mistakes more often. Second, outside factors somehow block strong splicing sites and by that ‘force’ spliceosomes connect with alternative weaker sites. And I discovered one pattern during my research that supports the second reason.
When the length of the introns is matter
Here goes an interesting tidbit! For many years scientists called introns ‘junk DNA’, because their functions were uncertain. However, now everything is upside down and clues to splicing regulation can also be found in introns. In case with PV larvae, introns around cassette exons are longer than those surrounding constant exons. Longer introns = more space for regulation elements = higher chances that some regulatory protein binds with a long intron and somehow affects splicing. For example, ‘force’ spliceosomes connect with alternative weaker sites.
I found 9 PV larvae proteins that potentially can bind with those long introns and up/down-regulate cassette exons. However, to make any critical conclusions about the role of those proteins in alternative splicing during the drying-recovery cycle of PV larvae needs more computational analysis and biological validation experiments.
Take-home messages
- Somewhere in Africa, ‘super-powered’ midge larvae (Polypedilum vanderplanki) can lose up to 97% of its body moisture and successfully survive in extreme environments. Isn’t it fascinating?
- One gene can code multiple forms of the protein, thanks to alternative splicing! Thus, evolution increases the biodiversity of proteins in eukaryotic organisms and helps them adapt to environmental changes.
- Alternative splicing significantly shifts by 3 major patterns in P. vanderplanki larvae during desiccation and recovery stages.
- Cassette exons are more often excluded from the sequence during the de/rehydration, while introns (or part of them) are retentained more frequently.
- I didn’t find any abnormalities of splicing sites around cassette exons in PV larvae and suppose that spliceosomes work the same way as for any other eukaryotic organism.
- Cassette exons are surrounded by longer introns in comparison with constant exons.
- I found 9 RNA-binding proteins that potentially participate in alternative splicing regulation during PV larvae extraordinary transformations.
Links
Original thesis:
Tagmazian, A 2020 ‘Alternative Splicing Regulation in Polypedilum Vanderplanki under Dehydration-rehydration Cycle’, Master thesis, HSE University, Moscow. https://www.hse.ru/en/edu/vkr/366647353
References:
Could be interesting to read:
More information about Polypedilum vanderplanki and its superpowers: https://www.naro.affrc.go.jp/archive/nias/anhydrobiosis/Sleeping%20Chironimid/e-about-yusurika.html